It’s a miracle: The $10-billion James Webb telescope was successfully launched, deployed, and activated. It’s now sending unbelievable pictures that you might have already seen:
The images are not just beautiful. They’re revealing the secrets of the universe. They’re charting uncharted territories.
Why does this matter? Because humanity is called by the stars, to visit them and bring them life. Because we’ll need space if we’re going to live forever. Because space is becoming an ever-increasing part of the human experience, defense, politics, and economy. We can’t understand the future of humanity if we don’t understand space.
And the core secret of space is encapsulated in this graph:
It explains why the Sun is as warm and yellow as it is. Why it doesn’t burn us. Why we stand on rocks and not gas. Why we see what we see, and not, for example, radio or wifi signals. How we can get almost infinite energy. What the James Webb telescope should be showing us. And much more. So how do you read this graph?
On the horizontal axis, you have the different elements in the universe (hydrogen, oxygen, carbon…) ordered by their size1 from the smallest (hydrogen on the left) to the heaviest (uranium on the right).
You can see it says “number of nucleons in nucleus”. A nucleon is a little ball of matter in the middle of an atom. There are two types, protons (which have a positive electric charge) and neutrons (without any charge). So for example hydrogen H1, on the left, only has one proton and that’s it. To the left, uranium has 92 protons and about 143 neutrons2 (which add up to 235 nucleons, hence the U235 name).
On the vertical axis, you have the stability of these atoms. You can see hydrogen (H1) all the way at the bottom left, which means it’s very unstable. The curve goes quickly up, then slows down, until it reaches the top with iron (Fe), the most stable of all elements. From thereon, elements become less stable again, all the way to uranium.
Stability is the opposite of energy. Something that has lots of energy is very unstable, and it tries to lose energy to become more stable3. So a nucleon in uranium has more energy than one of iron, and a nucleon in hydrogen has more energy than any other type of nucleon.
To understand stability in an intuitive way, imagine a bunch of little magnetic balls. When the magnets are separated and far away from each other, nothing happens. But when they get close to each other, they bundle up.
You can say that when these magnets are alone, they are unstable: at any time, they could stumble upon another magnet and immediately bundle up. Once they do, the combination is more stable but has less energy: now, you would need to apply some energy to force them to split4.
OK, so different elements have different levels of energy and stability. But how much of each element is there?
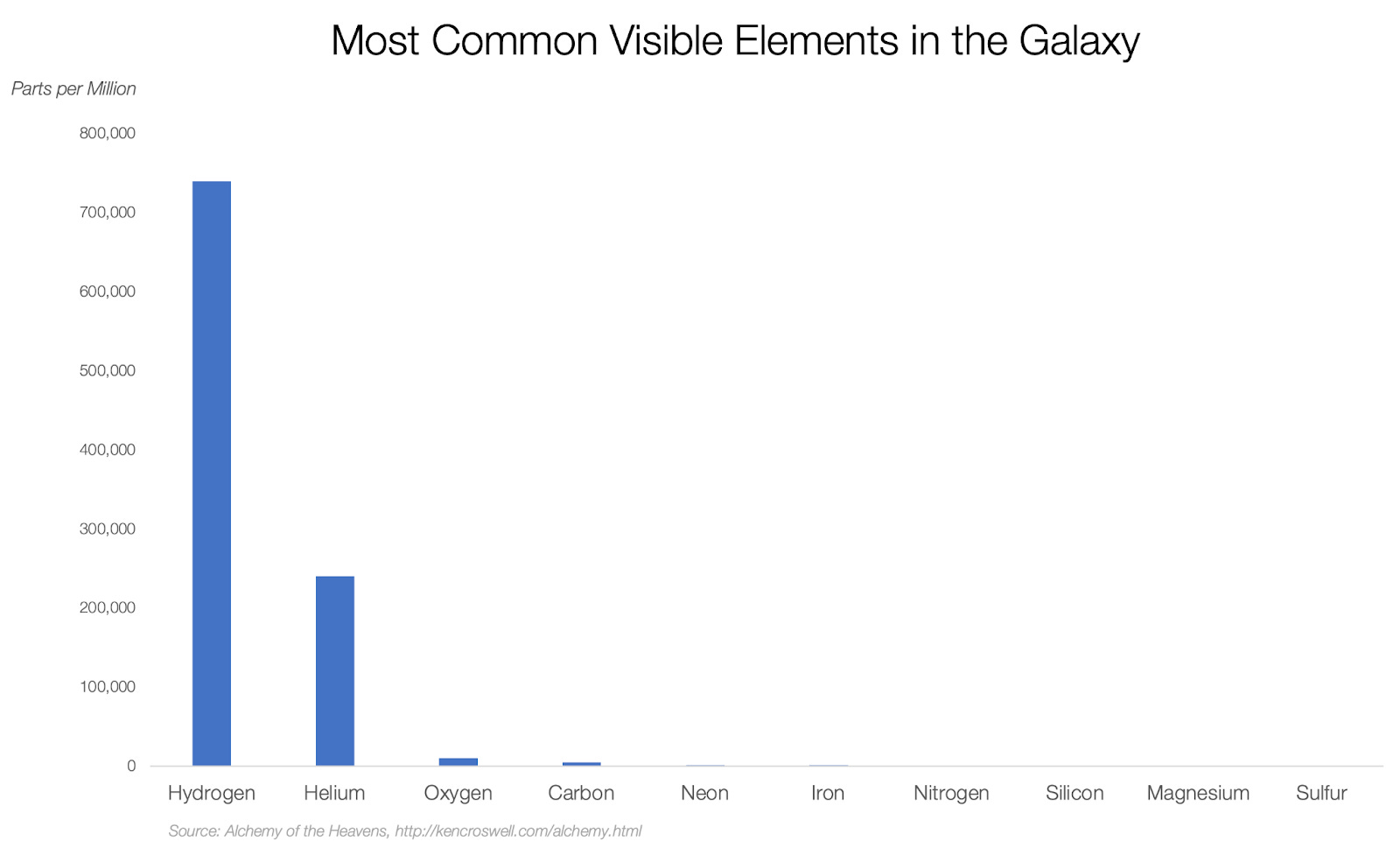
In fact, 98% of it is hydrogen or helium! Think about that: everything we have around on Earth, the nitrogen in our atmosphere, the carbon we’re made of, the silicon of sand, the iron and nickel of the Earth’s core… all of that is 2% of the matter in the galaxy!
How is this possible? Why is nearly everything hydrogen and helium? Because of the Big Bang.
It was pure energy. Energy concentrated into small particles, and as those particles collided with each other, they formed the smallest nucleons: protons and neutrons. Hydrogen is just a proton, so that’s why there’s so much hydrogen in the universe: it’s the simplest, most basic element, closest to pure energy.
Sometimes, during and just after the Big Bang, some of these hydrogen atoms combined with other hydrogens and neutrons to become helium (which is two protons and two neutrons).
This is not easy to do, however, because you might know that protons have a positive electric charge, so they repel each other. It takes a lot of energy to overcome that force, but once it is overcome, then these protons stick super strongly with each other5. They fuse.
If you go back to the metaphor of the little magnet balls, imagine they are scattered on a table. They are stable this way. Imagine that, for some weird reason, as you get two magnets close to each other, initially they repel each other. But if you force them to get close enough, suddenly you can overcome that initial repulsion, and a stronger attraction prevails6.
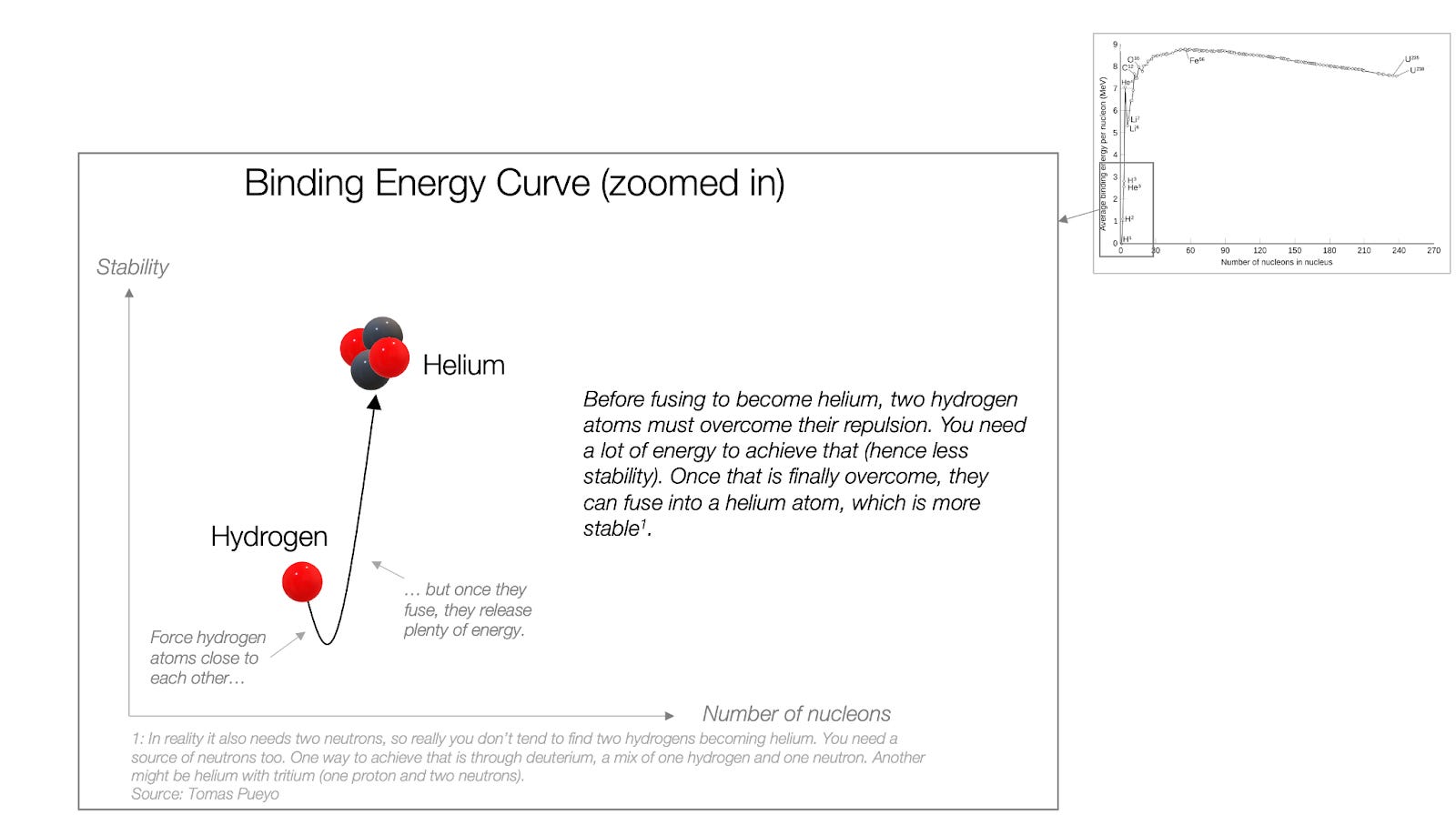
If you go back to the graph above, you’ll see that helium is much more stable than hydrogen. So when hydrogens and neutrons merge to become helium, they release a lot of energy in the process of becoming stabler.
But as you can see, protons need to become less stable before they can fuse with other protons and neutrons. That’s the energy required to vanquish the repulsion of positively-charged protons.
This process of protons and neutrons fusing with each other is called fusion, and you find it in two places: in stars, and in fusion reactors.
Star Fusion
This is the main process that happens in stars: hydrogen atoms fuse to become helium, releasing lots and lots of energy in the process. But why didn’t that happen in much greater quantities closer to the Big Bang? And how do stars gather the energy necessary to overcome the repulsion between protons?
In the minutes after the Big Bang, energy was very concentrated, so a lot of protons emerged, and some of them merged with neutrons to form helium.
But the density of energy was so high that all these particles spread in all directions. Within minutes, there wasn’t enough force to pressure them into helium. Most fusion stopped.
But then, through gravity, some of these protons and neutrons (=”nucleons”) started inching close to each other again. The more they came together, the more gravity was generated, and the more they attracted other nucleons. As they lumped together, pressure between them started increasing. At some point, there were so many of these nucleons together pressuring against each other that a few of them started fusing. The energy they released when fusing was enough to push neighboring nucleons to also fuse. The reaction chain ignited the entire lump of atoms.
A star is born.
A star is just enough matter together to pressure protons into overcoming their repulsion and becoming helium7, releasing a ton of energy in the process, which sparks further fusion of other hydrogen atoms.
This is what that reaction looks like in the most important curve in the universe:
This is the source of most energy from stars.
Let’s pause here for a second. Look at that massive jump in stability! To give you a sense of orders of magnitude, compare that with the process of fission.
Fission Energy Interlude
What we commonly call “nuclear energy” is fission. It splits Uranium into smaller elements.
When it splits into smaller elements, those elements are more stable than uranium, so the splitting releases energy. That’s what nuclear fission energy is made of.
You probably know how powerful it is, from nuclear bombs to the little combustible needed to power a massive nuclear plant.
Now compare it with fusion energy, fusing hydrogen atoms:
These are some of the reasons why everybody wants8 to discover fusion: it generates substantially more energy than splitting uranium atoms, and you can use hydrogen instead of uranium, which is extremely common. It’s everywhere.
End of fission interlude.
OK, let’s go back to our stars. We had just seen how stars transform hydrogen into helium, going up the stability curve, and releasing a ton of energy in the process.
If you follow the curve up and to the right, you can see that bigger elements than helium are even more stable. So with the right conditions, you should have helium merge into carbon, or nitrogen, or oxygen, or other elements… all the way to the most stable, iron.
Further, you should assume that the farther you go from helium, the less of that type of element you should have, because it’s harder and harder to make these fusions… except for iron, of which you should have a fair amount since it’s the most stable element. And beyond iron, elements should fall off a cliff in terms of abundance.
Indeed, this is what you see. Remember the graph above showing how much hydrogen and helium there is in the universe? The following graph is the same, but it shows all the elements. Since there are so few of them, it uses a logarithmic y axis instead of a linear one: an element that is at level 7 is 10 times more common than an element at level 6.
Remember how we said that hydrogen and helium together accounted for 98% of all matter in the universe? You can see it here: hydrogen (H) is at ~10, while carbon (C) is at 7, which means there are three orders of magnitude less carbon than there is hydrogen (so 1000x less).
What do we see looking at this graph? As expected:
The farther you go from hydrogen and helium, the less of other elements there are.
There’s much more iron (Fe) than if the graph simply continued going down. There is about 100 times more iron (Fe) than there is chromium (Cr), which comes before iron so should normally be more common than iron.
Elements after iron fall off much more quickly.
But there are some other weird things happening here:
Why are there so few atoms of Lithium, Beryllium, and Boron? As small atoms, shouldn’t there be more of them?
It seems like the curve follows a zigzag pattern rather than a straighter line. There’s a seesaw, where elements alternate between more and less frequent. Why?
Elements after iron fall quickly, but not to zero. How come there’s a reasonable amount of large elements that are less stable than iron?
Let’s investigate!
Since hydrogen is the least stable element, it will be the first to try to fuse into something more stable. It only needs the right conditions: a lot of hydrogen atoms very close to each other, some neutrons to help them stick together9, and a source of energy to overcome their repulsion.
This is what stars do: gravity brings many hydrogen atoms together and compresses them a lot. Some of them fuse, releasing a lot of energy. That energy is captured by other hydrogen atoms that also fuse and also release energy. This is why stars mostly burn hydrogen into helium.
But here’s something else that’s happening inside stars: at the same time as gravity is pulling these atoms together, all this energy emitted is blasting them apart. Imagine all these hydrogen and helium atoms smashing into each other all the time: they’re pushing each other apart! And the energy that is released during fusion also pushes all these elements apart.
The size of a star like the sun is the direct result of an equilibrium between these two forces: the gravity pull and the energy push.
Once a star has burned most of its hydrogen and is made up of mostly helium, it enters another phase.
On one hand, helium is heavier, so its gravity is stronger.
On the other hand, fusing helium into other elements generates less energy: we’re higher up in the stability curve.
Fusing helium creates carbon (three helium atoms for one carbon), but that fusion reaction creates much less energy than the one from hydrogen to helium.
Since more helium means more gravity, and more fusion of helium into other elements means less energy pushing atoms away, the star compresses. It becomes smaller. This added compression increases energy, which makes sure that the star stays alight, but merging helium now instead of hydrogen.
This process then continues after all the helium is burnt, going up and to the right in the stability curve.
Rare, Light Elements
But there’s so little lithium (3 protons), beryllium (4 protons), and boron (5 protons), as we saw. That’s because they can’t be formed by fusing helium. Why?
The most common helium is Helium 4: two protons and two neutrons. This helium is more stable than lithium, beryllium, or boron.
Once you have helium, going to lithium, beryllium, or boron results in less stability. So that doesn’t happen10. Instead, what happens is that three heliums fuse into a carbon, which is stabler than helium, and in the process it releases energy.
Zigzag
Add another helium to carbon (six protons), and you get oxygen (eight protons). Another, and you get neon (10 protons). And so on and so forth. And this is one of the reasons why you see a zigzag shape in the frequency of elements.
Usually, stars don’t mix all the elements all the time. When they have enough hydrogen, that’s what they burn the most. But once they’ve finished all their hydrogen, some start fusing their helium into carbon, and so on and so forth. So hydrogen atoms mostly fuse with other hydrogen atoms, forming helium. Once helium is the only thing left, it starts fusing.
Since helium has two protons, you get more elements with an even number of protons than with an odd number of them. For example, carbon has 6 protons: it’s the fusion of three heliums. Oxygen has 8 protons: carbon plus one helium.
Meanwhile, nitrogen (N) has 7 protons, fluor (F) has 9, sodium (Na) has 11. These are odd numbers, and they’re less frequent than their even partners. One of the reasons is because they require one additional proton to be added.
But there are always protons available, even in stars that have exhausted most of them. So this alone can’t satisfactorily explain the jagged shape of this curve, all the way to the end. What gives? The reason is atomic structure. It turns out that atoms are also more stable when they have pairs of protons and pairs of neutrons11. As a result, throughout the entire curve, elements with pairs of protons are about 10x more common than those of approximately the same size but with an odd number of protons.
Bigger Atoms than Iron?
After burning all the hydrogen, the more mass a star has, the higher the temperature, and the more types of atoms will be fused in the core, all the way to iron for very massive stars.
Beyond iron, nuclear reactions don’t create energy, they consume it. That makes them much, much less frequent. But they’re still possible because there’s so much mass in such a tiny place that atoms still find each other. For example, an iron can still find a helium and fuse into nickel. That will consume energy, but there’s a lot around anyway, so atoms bigger than iron can be formed that way12.
This chart summarizes the sources of every atom type.
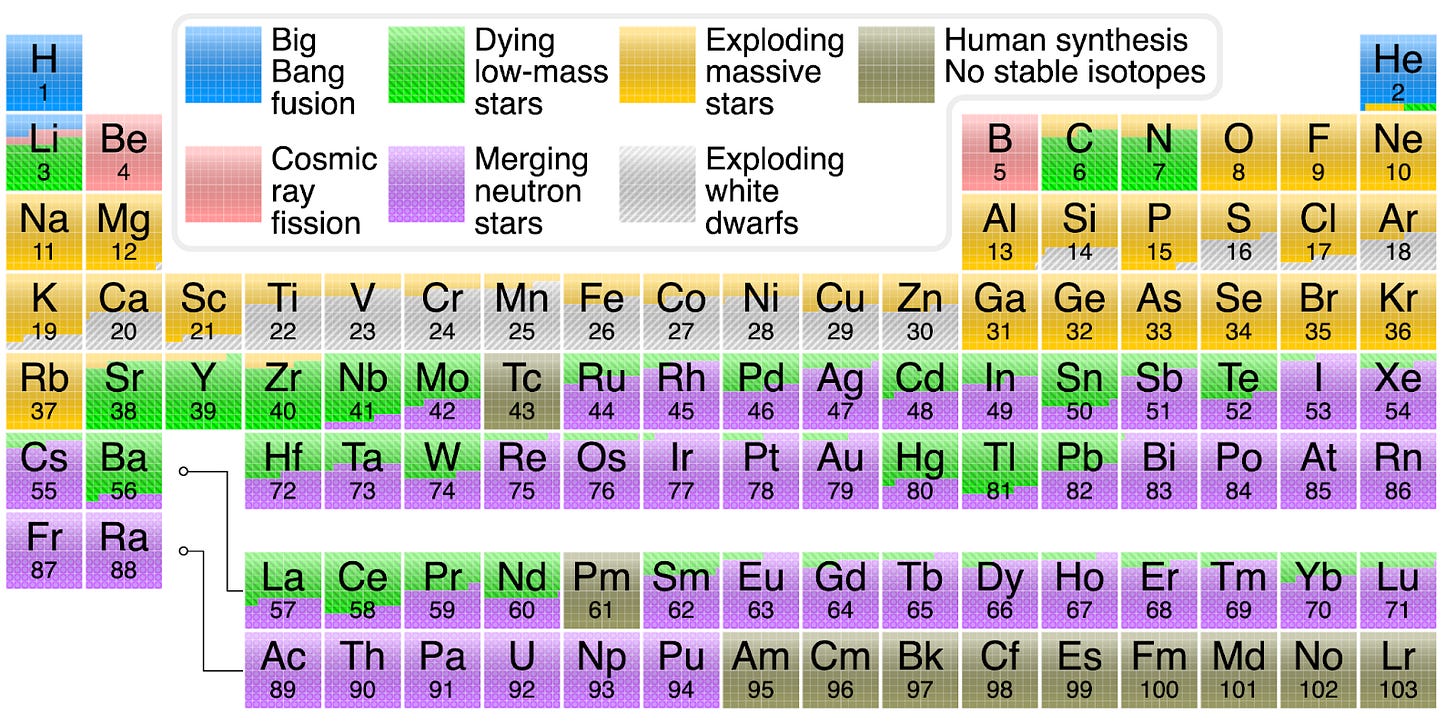
As you can see, hydrogen and helium were created mostly during the Big Bang (and a bit of lithium). Some helium was formed afterwards, in stars. After that, you have to jump to carbon to start getting new atom formation in stars, because beryllium and boron can’t be formed there.
But as you can see, there are other sources for some elements. It turns out that when stars explode, a massive amount of energy and lots of fusion happens at once. These newly formed elements, along with the rest of elements of the star, are shot across the universe in such explosions.
What happens then?
Keep reading with a 7-day free trial
Subscribe to Uncharted Territories to keep reading this post and get 7 days of free access to the full post archives.